Drip, trickle, microemitters, and subsurface irrigation systems are considered low-volume irrigation. Low-volume irrigation systems are designed to improve irrigation efficiency, delivering water to the crop accurately with minimal water loss. Irrigation efficiency (Table 1) can be categorized into two main concepts: water loss and uniform application. If water loss is significant, or application uniformity is poor, efficiency will be low. Generally, the most significant loss of irrigation water is from overwatering, where the water percolates below the root zone, or from runoff. With good management, losses due to leaks, system drainage, and flushing of filters and lateral lines should not exceed 1%. Low- volume systems have the opportunity to achieve efficiency, and under careful management, will minimize losses from overirrigation. However, using low-volume systems requires increased irrigation frequency and soil moisture monitoring should be used to improve water-use efficiency.
Uniformity is desired for each application of water, meaning that the irrigated area receives the same portion of water throughout. A well-designed irrigation system accounts for variability by applying water based on the soil structure (e.g. sandy, silt, loam, clay, and organic matter) and environmental conditions (e.g. wind, temperature, and cloud cover). That said, systems can have losses inherent to the design (Table 1). There may be variability in efficiencies that are noticeable even when comparing low-volume emitters (i.e. microemitters) compared to point-source emitters (Table 1). Low-volume irrigation systems are highly controlled, need constant attention, and will not sufficiently recover a soil water deficit without the potential of crop loss. In comparison to an overhead sprinkler system, low-volume systems are a challenge to keep up with water demand if regular irrigation is not scheduled and maintenance is not performed.
IRRIGATION SYSTEM EFFICIENCIES | |
Surface irrigation | Attainable Efficiencies |
Basin | 80 - 90% |
Border | 70 - 85% |
Furrow | 60 - 75% |
Sprinkler Irrigation | |
Hand or portable | 65 - 75% |
Traveling gun | 60 - 70% |
Pivot: center or linear | 75 90 % |
Solid set or permanent | 70 - 80% |
Low-volume Irrigation | |
Point-source emitters | 75 - 90% |
Line source | 70 - 85% |
Subsurface | 85 - 95% |
Microemitters | 70 - 80% |
Source: Rogers et. al., 1997
Low-volume systems
All low-volume systems are similar up to the lateral lines where the water emitters are located. Figure 1 is a diagram of a low-volume irrigation system. The system starts at a water source, which can be well, surface,municipal, and/or rainwater collection pond. Regardless of the source of water, there needs to be a force or pressure that pushes the water through the lines and to the crop.
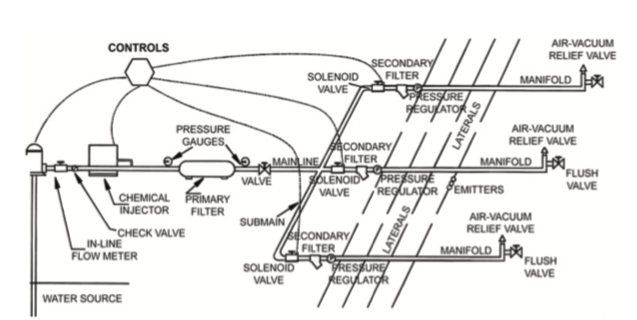
Water pressure
The most rudimentary water pressure system is gravity. A gravity system is dependent on open-head pressure applied through gravitational force by elevating the water source above the crop. Calculating line pressure is based on the height of the reservoir above the highest point to be irrigated by a factor of 0.433.
Height of reservoir x 0.433 = pounds per square inch (psi)
50’ x 0.433 = 21.7 psi
Usually, gravity systems are used on a small scale and the collection vessel should have the capacity to supply one application of irrigation water. Water weighs approximately 8.3 pounds per U.S. gallon and constructing a gravity irrigation system should account for the amount of water needed by the crop. Irrigation water demand for a mature blueberry plant can range from 0.5 to 2 inches per week per acre dependent on canopy size, soil type, and weather. An acre-inch of water equals 27,154 gallons. If your planting is a quarter-acre of production area, the capacity of your tank should be 6,789 gal/7 days with consideration of the loss of efficiency (e.g., 15% loss through drip irrigation), which equals 1,115 gal for each day’s demand at 1 acre-inch of water per week. For this example, the reservoir should be able to hold 4.6 tons of water elevated at 35 feet above the crop to supply 15 psi. Another way to think about water demand for blueberry is on a per plant basis where a mature actively growing plant can use from 1.5 to 4 gallons of water per day, dependent on canopy size, soil type, and weather.
Other systems used to deliver system pressure are mechanical, using either an electrical motor or combustion engine
as the prime mover to operate the pump. Choosing a delivery system to apply pressure to the water should be based on the efficiency of the energy being used to drive the system. To evaluate a system’s efficiency, the grower should have a reasonable estimation of production acreage, the number and size of zones to be irrigated, and the source of energy to drive the system (electricity, diesel, gasoline, natural gas, propane, or other). Efficiency is dependent on the cost to deliver an acre-inch of water, which is defined by the work produced per unit of energy, the distance the water is lifted from the well or surface water to the outlet of the pump, discharge pressure, performance rating, and energy unit cost.
The capacity of an irrigation system is dependent on the size of the production area. Blueberries that are planted in rows are spaced to provide access for making chemical applications, pruning, general maintenance, and harvest. Row width is dependent on the machinery used to access the plants, and generally, 12-foot spacing between the rows is sufficient. Blueberries are generally bedded at 4-feet wide by 18-inches high at the crown of the bed. Plant spacing in the row is 4-5 feet for rabbiteye blueberries and 1.5-3 feet for southern highbush blueberries. There are many options when selecting the type of emitter system to deliver irrigation water, but the amount of water and the irrigation run time are critical to deliver adequate water for high-quality fruit. System demand should be calculated for peak demand, which could be at 2 acre-inches of water per week for mature plants under high-heat, low-moisture conditions in sandy soils. If the drip line delivers 0.24 gpm/100 feet, bed spacing is 12 feet x 100 feet of row, and with drip line efficiency at 85%, the demand on the system per acre will be as follows.
Using 2 acre-inches per week, what is the water demand on a daily (d) basis per acre (A)?
(54308 gal/A)/ 7 d = 7758.3 gal/A/d or 2 in/7 d = 0.29 in/A/d
At 85% efficiency, the water demand per acre per day is:
8922 gal/A/d (GPAD)
How many 100-foot rows in an acre at 12-foot spacing?
(43,560 ft2/1 A) x (1 A/100 ft) x (1/12 ft) = 36.3 (100-ft rows/A)
Gallons applied per 100 feet.
8922 GPAD/36.3 (100 ft/A) = 245.8 gal/100 ft
Pump demand for 100 A:
100 A x (8.7 gum/ 1 A) = 870 gpm
If the system has a 100 gym pump, how many zones are there?
870 gpm/100 gpm = 8.7 zones
Acres per zone:
100 A/8.7 zones = 11.5 A/zone
Time to irrigate a zone:
(245.8 gal/100 ft) x (100 ft/0.24 gpm) = 1024 min or 17.1 hrs
The longest recommended time for a pump to continuously run is 15 hours or less, and with a required run time of 17.1 hours, the plants will not receive sufficient water at 2 acre-inches of demand for this system. Both the gpm of the drip line and the pump capacity should be increased to meet demand. If 0.4 gpm/100 feet drip line was used, then the run time for one zone will equal 10.4 hours. There are around nine zones to irrigate in a day, so increasing pump capacity, increasing well diameter, adding irrigation water sources, and increasing flow rate of the drip line will be needed to provide sufficient irrigation under the most stressful growing conditions.
Irrigation costs are directly related to the pumping lift (location of the water source relative to the elevation of the pump discharge or the static water level plus the drawdown for a well) and the cost of energy to create the lift, which is determined by the area to be irrigated and crop water demand. Calculating the estimated water usage of the crop under the greatest demand will identify the pumping lift needed to fulfill demand. As mentioned before, there are various options to provide energy to the pump. Table 2 includes comparisons of energy options based on average energy content, prime mover efficiencies, pumping plant efficiencies (a ratio of the work accomplished compared to the energy available in the fuel), and the Nebraska Pumping Plant Performance Criteria (NPPPC). The NPPPC is a long-term study of well- water irrigation pumping system design and management to calculate the average amount of work derived from a unit of energy in a well-designed and managed irrigation system. This work provides criteria for average efficiencies to which a working system can be compared so that losses can be identified and corrected to minimize unnecessary expenditures occurring on the farm.
Average energy content | Nebraska pumping plant performance criteria | Engine or motor efficiency (%) | Pumping plant conversion (%) | |||
---|---|---|---|---|---|---|
Energy source | BTU | Horsepower (hour) | Engine or motor performance (hp-hr/unit) |
Pumping plant performance (who-hr2/unit3) |
||
1 gallon diesel fuel | 138,680 | 54.5 | 16.7 | 12.5 | 31 | 23 |
1 gallon gasoline | 125,00 | 49.1 | 11.5 | 8.66 | 23 | 18 |
1 gallon liquefied petroleum gas (LPG) | 95,475 | 37.5 | 9.2 | 6.89 | 25 | 18 |
1 therm natural gas | 100,000 | 39.3 | 8.06 | 6.05 | 21 | 15 |
1 kilowatt-hour of electrical energy | 3,412 | 1.24 | 1.18 | 0.885 | 88 | 66 |
1 Conversions: 1 horsepower (hp) = 0.746 kilowatts (kW), 1 kilowatt-hour (kW-h) = 3412 BTU, 1 horsepower-hour (hp-h) = 2,544 BTU Source: Martin et al., 2011 |
The amount of energy required for delivery of an acre-inch of water from a well-designed and maintained system by calculating from pumping lift and discharge pressure is shown in Table 3. A system that has a pumping lift of 350 feet operated at 20 psi uses 3.61 gallons of diesel fuel based on a performance rating of 100%. If the system is operating at 80% of the NPPPC rating, the fuel usage will be 4.5 gallons of diesel (3.61 x 1.25, the multiplier from Table 4). To identify the energy needed to apply an acre-inch of water, use the conversion factors listed in Table 5 to calculate the other energy sources listed in Table 2.
PUMP DISCHARGE PRESSURE
LIFT (FEET) | PUMP DISCHARGE PRESSURE | ||||||
---|---|---|---|---|---|---|---|
10 | 20 | 30 | 40 | 50 | 60 | 80 | |
0 | 0.21 | 0.42 | 0.63 | 0.84 | 1.05 | 1.26 | 1.69 |
25 | 0.44 | 0.55 | 0.86 | 1.07 | 1.28 | 1.49 | 1.91 |
50 | 0.67 | 0.88 | 1.09 | 1.3 | 1.51 | 1.72 | 2.14 |
75 | 0.89 | 1.11 | 1.32 | 1.53 | 1.74 | 1.95 | 2.37 |
100 | 1.12 | 1.33 | 1.54 | 1.75 | 1.97 | 2.18 | 2.60 |
125 | 1.35 | 1.56 | 1.77 | 1.98 | 2.19 | 2.4 | 2.83 |
150 | 1.58 | 1.79 | 2.00 | 2.21 | 2.42 | 2.63 | 3.05 |
200 | 2.03 | 2.25 | 2.46 | 2.67 | 2.88 | 3.09 | 3.51 |
250 | 2.49 | 2.7 | 2.91 | 3.12 | 3.33 | 3.54 | 3.97 |
300 | 2.95 | 3.16 | 3.37 | 3.56 | 3.79 | 4.00 | 4.42 |
350 | 3.4 | 3.61 | 3.82 | 4.03 | 4.25 | 4.46 | 4.88 |
400 | 3.86 | 4.07 | 4.28 | 4.49 | 4.7 | 4.91 | 5.33 |
Source: Martin et al., 2011
RATING (%) | 100 | 90 | 80 | 70 | 50 | 30 |
Factor | 1.00 | 1.11 | 1.25 | 1.43 | 2.00 | 3.33 |
ENERGY SOURCE | UNITS | FACTOR1 |
Diesel | gal2 | 1.00 |
Electricity | kW-h3 | 14.12 |
Propane | gal | 1.814 |
Gasoline | gal | 1.443 |
Natural Gas | thm4 | 2.066 |
1Factor is calculated by dividing pumping plant performance number of diesel by the pumping plant performance number of the other energy source (Table 2; ex. 12.5 (diesel)/0.885 (electricity) = 14.12 kW-h3) 2gal = gallon |
Comparing electric to diesel, with 350 feet of lift and 20 psi (assumption of $2.50 /gal for diesel and $0.10/ kW-h),
multiply diesel use at 100% performance by the electrical conversion factor (Table 5)
3.61 x 14.12 = 50.97 kW-h
3.61 gal of diesel x $2.50 = $9.03/acre-inch
50.97 kW-h x $0.10= $5.10/acre-inch
The efficiency and demand calculations are practical for estimating the cost of a system, but when ultimately designing and deploying the system, it is recommended to get the advice of an experienced irrigation specialist. The authors advise knowing federal, state, and local regulations on water use, whether taken from surface or subsurface sources. Contact your county Agriculture and Natural Resources Cooperative Extension agent for further information and contact information.
Components of a low-pressure irrigation system
Backflow prevention
Backflow prevention devices protect the water source and are mandatory in Georgia if applying chemicals such as, but not limited to, fertilizers and pesticides. Laws and regulations on antisyphon or backflow devices can be mandated by federal agencies to local municipalities, so knowing the type and use of antisyphon or backflow prevention devices is important for compliance.
Backflow prevention can be as simple as an air gap between the source and the outflow. However, most systems require pressure and are closed from the irrigation water source to the crop. In a closed system, a device that stops water from siphoning back into the source must be installed if applying chemicals and plant nutrients. There are a number of options, but ultimately the choice of backflow device may have been mandated by a regulatory agency. Know the law and requirements concerning the design of your irrigation system. The following describes some available backflow devices.
-
An atmospheric vacuum breaker (AVB, Figure 2) is an inexpensive backflow preventer. The AVB is installed after each control valve that isolates the source from the system. As water pressure from the source drops, so does the poppet valve in the AVB. If a valve is closed to the outflow side of the AVB, then the atmospheric pressure that creates the vacuum will be lost—and so will the backflow protection. The AVB must be installed 6 inches higher than the highest point from which water is applied (Figure 2)
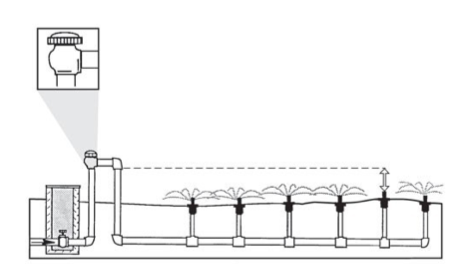
-
Antisiphon valves are manual or automatic controlvalves with a built-in AVB. Like an AVB, the antisiphon valve must be installed 6 inches higher than the highest point from which water is applied. Antisiphon valves are inexpensive and commonly used in residential irrigation systems. Also, like AVB, antisiphon valves should be installed after the last control valve to the irrigation emitters.
-
A pressure vacuum breaker (PVB, Figure 3) is similar to AVB except only one unit needs to be installed between the mainline and the control valves. Like AVB, the PVB must be installed 6 inches above the highest point from which water is applied. PVB, AVB, and anti-siphon valves may leak from the device when water pressure is shut off. Mount these valves in an area where spillage is not a concern. Further, PVB valves may spill each time the water is shut off, especially when a well pump is operated in tandem with a pressure tank. A spring-loaded check valve may reduce or eliminate spillage from PVB valves.
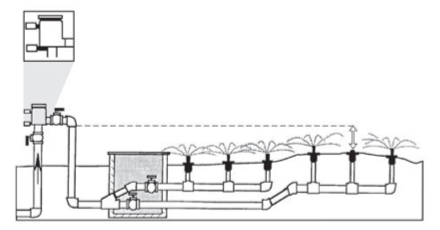
-
A reduced pressure backflow preventer (RPBP, Figure 4) is constructed to keep hazardous materials from contaminating water sources. This type of device is used in commercial irrigation systems and can be fairly expensive. A single RPBP is installed before all valves and must be at 12 inches above the ground but not above the highest point from which water is applied. These units may spill water if backflow is detected or if broken.
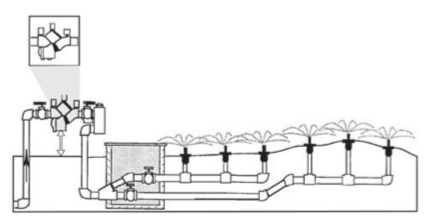
-
A double check backflow preventer (DCBP, Figure 5) involves two spring-loaded check valves mounted in series with shut-off valves installed at either end of the valve. This allows the DCBP valve to be isolated so that operational tests can be conducted on the device. The DCBP does not have to vent air or spill water when backflow occurs. The DCBP does not need to be elevated and may even be installed underground. However, there may be restrictions on the use of DCBP valves, so check local regulations before installing.
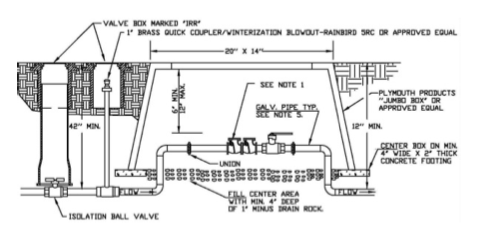
-
A chemigation check valve (CCV, Figure 6) is the most common antisiphon device used in agricultural irrigation (Figure 6). The CCV has a spring-loaded positive pressure closing flapper. The two bolts on the top of the CCV identify the location of the valve. As part of the CCV, a vacuum relief valve is manufactured into the design to relieve the air hammer and serve as an inspection point. Below the vacuum relief valve is a low-pressure drain, and the CCV has a drain plug.
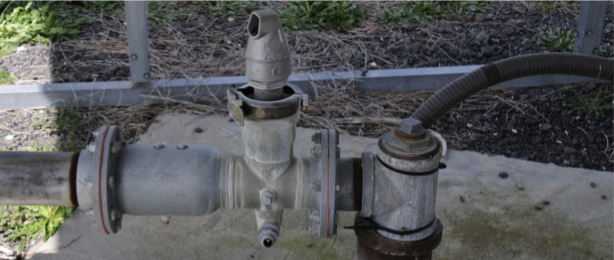
Monitoring water flow
Measuring water flow is necessary for effective water management. Water flow can be measured through a number of devices, and the device should measure instantaneous quantity and record total quantity. Instantaneous quantity is the rate at which water is flowing, for example, gallons per minute (gpm). Total quantity refers to how many units of water have passed the meter in a specific time period. The standard unit will be in gallons but special-ordered meters may read acre-feet. If large volumes of water are being applied, use a meter that reads in thousands of gallons to tens of thousands of gallons.
The meter should be matched to the system. To ensure that the proper meter is used, familiarize yourself with:
-
The range of flow the system operates. If pumping 20 gpm for one set and 90 gpm for another set, the meter must be able to measure volumes over this range. Install units that have been certified and can be tested for accuracy periodically.
-
The pipe dimensions where the flow meter will be installed.
-
The manufacturer’s specifications for installation. Some meters may require a specified straight length of pipe before and after the meter to reduce nonuniform laminar and turbulent flow.
-
The irrigation water quality. If water is hard, caustic, and/or contains particulates, meters with moving parts can eventually be corroded, impaired, and worn prematurely.
Flow meters may measure flow through mechanical operation or without moving parts. The most common type of meter is a propeller-type device (Figure 7), which spins as water passes. Propeller revolutions are counted to measure rate and volume. The flow rate is a function of rotational speed, which can be monitored electronically or mechanically. Gear- driven devices are used where electrical monitoring is not practical. Propeller meters are subject to bearing wear. In addition, turbid, hard, and alkaline water can cause excessive wear to the propeller, drive shafts, and bearings. Regular maintenance should be scheduled for gear-driven meters.
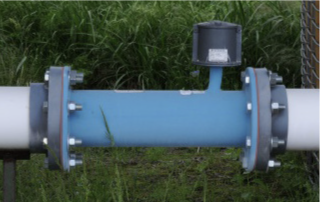
Vortex meters measure eddies or vortices created by placing an object into the pipe and disrupting the flow stream. Electromagnetic flow meters measure an electric field created by a liquid moving through a magnetic field. Both types of meters require electricity and may be either an inline flange fitting or insertion device.
Flow meters are critical tools used to manage nutrient and pesticide applications to the crop by monitoring water flow. Here is a simple calculation that converts gpm to acre-inches of water:
pump gpm/449 gpm = inches of water applied to an acre in one hour
Example:
(100 gpm/1 hr) x (1 acre inch/449 gpm) = 0.22 acre inches/hour
Fertilizer and chemical injection
It’s common practice to apply chemistries through a low-pressure irrigation system. Two terms are used to describe the type of chemistry being applied through irrigation water. Chemigation is used to describe the application of chemicals such as pesticides or system maintenance compounds (sanitizers, line cleaning chemicals, and pH adjusters). Fertigation is used to describe the application of plant nutrients and soil amendments. Chemigation is regulated by the Federal Insecticide, Fungicide, and Rodenticide Act (FIFRA). The U.S. Environmental Protection Agency (EPA) enforces FIFRA and authorizes which pesticides can be used in an irrigation system. If a product is authorized for chemigation use, the product label will contain instructions for proper use.
Injectors introduce fertilizers and other chemicals into the irrigation system. The least expensive injectors are the venturi- type. Venturi injectors work with the system water pressure where a constriction in the pipe increases the flow, which creates a vacuum and draws the solution into the irrigation water. Another common injector is a positive displacement injector that also works on water pressure. This system introduces the solution into the irrigation water in metered doses. Water pressure in a low-volume irrigation system at the injector should be ~20 psi and the injection system should operate optimally at this pressure.
Both the venturi and positive displacement injectors work by drawing a solution from a vessel into an irrigation line. Working and handling fertigation and chemigation solutions requires some level of personal protective equipment (PPE) to minimize exposure. At a minimum, wear a long sleeve shirt, long pants, shoes and socks, chemical-resistant gloves, and protective eyewear. Additional PPE may be required when dismantling injectors for maintenance and repair. Furthermore, when applying any injectable chemistry, check the label for the required PPE to handle the product safely. If applying acids to irrigation water, face shields should be worn in addition to required PPE to minimize exposure.
There are other options for chemical injection that require mechanical or electrical inputs to operate the injector. Choose a serviceable system that can meet operational demand and is weather- and chemical-resistant. For more information on chemigation, see UGA Extension Bulletin 1298, “Chemigation in Georgia.”
Filtration
Irrigation water should be filtered. Particulates can clog emitters, which will cause skips in the irrigation pattern and can reduce yield. The type and intensity of filtration depends on the water source. If using surface water, use screens on the inlet pipe. The inlet pipe (with a screen attached) should not be set on the bottom of the reservoir. There should be a minimum of 6 inches between the bottom of the reservoir and the inlet. This will aid in preventing foreign matter from clogging the screen and entering the irrigation system. A sand filter (Figure 8) is highly recommended if using a surface water source. If the water source is dirty with high levels of particulates, organic matter, and other debris, a sand filter can remove larger particulates. Sand filters can have manual or automatic back-flushing systems for cleaning the filter. Ponds with algae growth and other particulates will cause sand filters to quickly clog, requiring frequent backflush events.
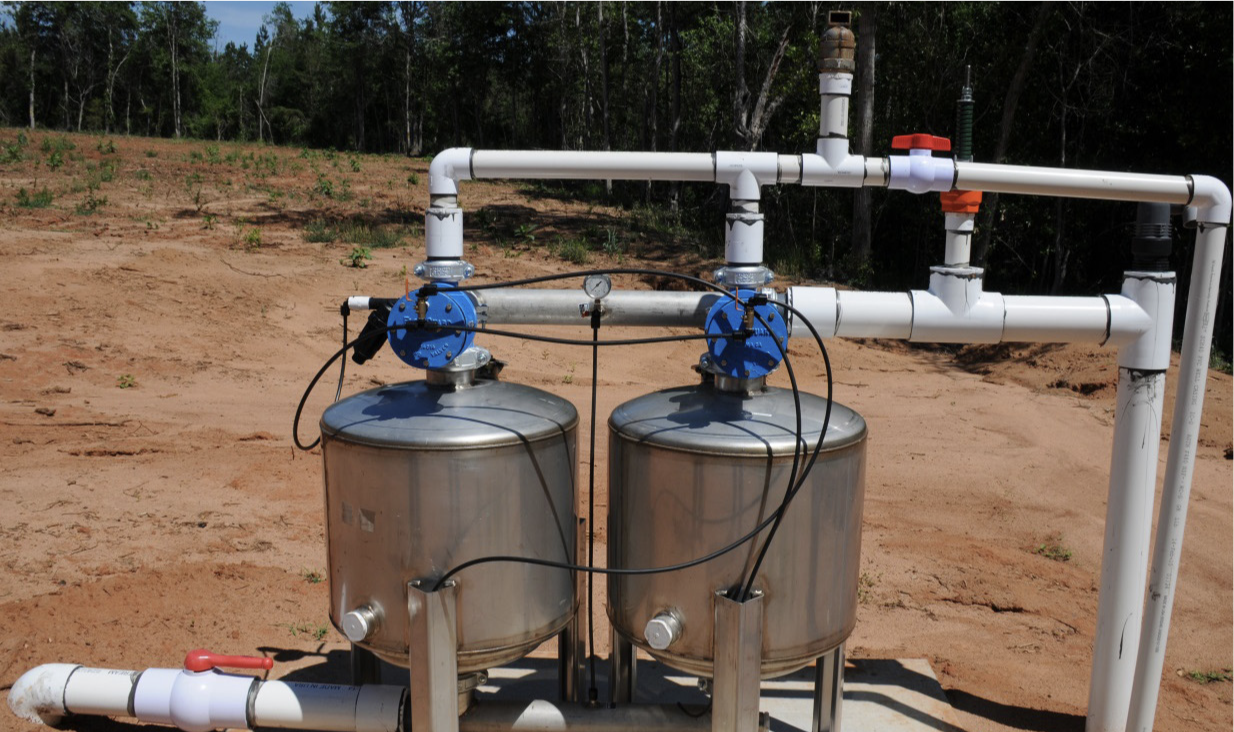
Well water shoulured be tested before use in the irrigation system. Water hardness, dissolved solids, iron, and manganese can all clog an emitter (see UGA Extension Circular 1105, “Blueberry Irrigation Water Quality”). Furthermore, some wells may have substantial particulates, and the water should be pumped through a sand filter before being introduced to the system. Regardless of irrigation water source, water entering the laterals should pass through fine filtration, either cartridge or screen filters of 80 to 200 mesh depending on the emitter type and water delivery volume.
System controls
This section covers monitoring, isolation, pressure regulation, solenoids, controllers and safety devices in a low-pressure irrigation system. Pressure gauges are important to identify if the system is properly working. Fixed pressure gauges should be mounted before and after filters (sand and final filters). If the pressure reading before the filter is higher than the pressure reading after the filter, this is an indication that the filters are clogged and should be cleaned. Gauges can be mounted in other locations to identify whether isolation and pressure regulation valves are working correctly. Fluid-filled gauges are suggested because they reduce needle vibration and are less likely to be internally damaged.
Valves are available in metal (brass, stainless steel, and iron) or plastic/PVC. Valves are used to isolate zones, irrigation components (filters, injectors, pumps), and drain lines. Valves are rated for pressure, sunlight exposure, and chemical resistance. Manual valves are manufactured in two basic designs, gate or ball valves. Although there are various configurations, inexpensive gate valves tend to fail and ball valves have greater durability. Light exposure should also be considered, as some plastic valves are destroyed by UV light.
Solenoid valves (Figure 9) are used with a controller to automate the irrigation system. Solenoid valves use electricity to operate, powered either by batteries or 24-volt alternating current. The valves have two basic styles: globe or angle valves and anti-siphon valves. Globe or angle valves do not incorporate an antisiphon device, and if injecting chemicals or plant nutrients, a backflow device must be installed. For an antisiphon solenoid valve to work, it must be installed 6 inches above the highest irrigation emitter. Controllers vary by manufacturer and compatibility of valves to the controller should be thoroughly researched before purchasing. Electrical wires are commonly chewed by animals and installing solenoid valves in an enclosure should reduce damage.
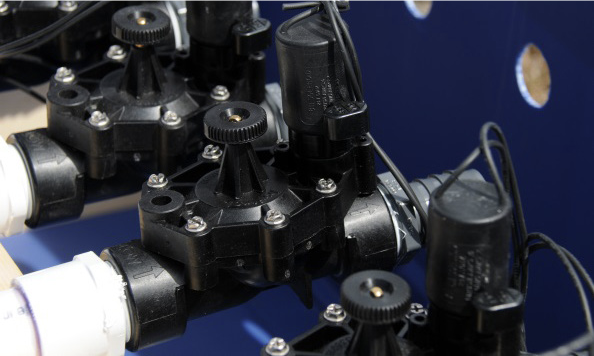
An advantage of low-pressure irrigation is that the pump pressure at field entrance can be as low as 20-25 psi and 10-12 psi at the laterals. In comparison, overhead irrigation systems use 50-70 psi. Pressure regulation valves are used to reduce pressure and may be installed at each zone. Without pressure regulation, drip tubing and emitters may be damaged by overpressuring the laterals.
Laterals: applying irrigation water
Laterals (Figure 1) are the parts that deliver irrigation water to the plants. There are two general categories of laterals for low pressure irrigation, drip and microsprinklers. Drip irrigation systems use inline emitters. Though there are other options, growers tend to use 0.24 to 0.50 gpm flow rates with emitter spacing at 12 to 18 inches. However, careful consideration of the dripline configuration should include plant spacing, soil type (with closer spacing for sandy soils), and the size of the irrigation block. For plantings in areas that have sandy soils or are prone to drought, laying two drip lines per bed may improve irrigation. When establishing a planting, many growers may fumigate and/or lay plastic mulch, where the plastic will be placed over the drip line and bed (Figure 10). For beds covered with plastic mulch, microsprinklers are not suitable for irrigation and point-source emitters are time-consuming and difficult to install.
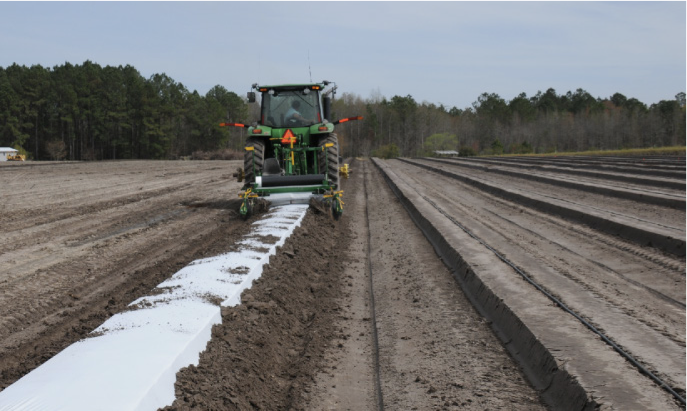
Microsprinklers (Figure 11) are also considered low-volume irrigation but differ from inline drip emitters because the lateral line doesn’t have perforations. Each microsprinkler must be installed by hand by punching holes into the line to connect the microsprinkler to the irrigation water. Microsprinklers are available in many configurations of spray patterns, flow rates, and mounting systems. Microsprinklers are less efficient at applying water than drip systems (Table 1) because they provide a greater wetting area. Microsprinklers have been adopted by orchardists, especially for crops grown in sandy soils.
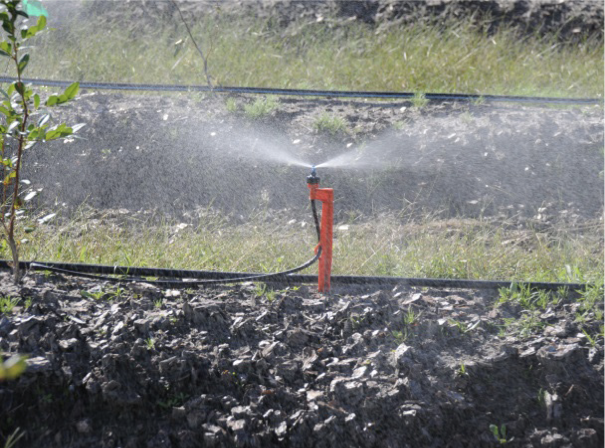
Blueberry roots are fine and are concentrated within the top 18 inches of soil. Microsprinklers can provide irrigation water over a larger area and can effectively keep soil moisture above the wilting point, apply more water faster than drip, and may minimize effects of long, dry periods to positively affect blueberry production. Microsprinklers have larger orifices than inline drip emitters and may minimize clogging if the water quality is poor. Because the microsprinklers are positioned aboveground, it is easier to identify clogs. However, microsprinklers are prone to damage from UV radiation, exposure to heat and cold, harvesting (hand and mechanical), and other management practices. With a greater wetted area, weed germination increases, and under windy conditions the fine irrigation water mist can be blown off-target. Microsprinklers are useful irrigation tools and like any management decision should be considered carefully.
Preparing to construct a low-pressure irrigation system should include making measurements to identify the quantity of materials needed to complete the system. Identify the length of runs: main, submain, and lateral. Prepare a schematic that accounts for bends and tees. From the plan, make a material list to serve as inventory and to estimate cost. Items to consider are the pumping system, pipe/tubing, connectors, appropriate glue or cement, hold down stakes (drip line will walk off the bed through heating and cooling), labor, and the tools to accomplish the task. It is also important to carefully consider control zone size during the planning phase. Control zones are to be designed based on the water supply and field size. The control zones should be sized such that the required amount of water as calculated earlier in this publication can be applied within system specifications. It is important not to overdemand the irrigation system, and ensure that the system has the capacity to irrigate the zones without running the system continuously.
Using the chemigation system
Chemigation is a term used to describe the addition of chemicals to irrigation water. This includes pesticides, fertilizers (fertigation), acids, and drip line sanitizers (chlorination). A properly designed chemigation system will have a facility that reduces human and environmental exposure to agricultural chemicals. Chemistries are brought to the farm in a container that will need to be stored, handled, and disposed of safely. These areas and structures are called secondary containment, which is designed to prevent spills from contaminating the environment and minimizing any impacts to human health. UGA Extension Bulletin 1095, “Pesticide Storage and Mixing Facilities,” covers the pertinent terminology and designs, but requirements and design may be regulated. Check with local authorities to satisfy the proposed rules and regulations.
With all the requirements in place, applying chemicals through an irrigation system is dependent on the label recommendations of each product. Chemistries that are applied as solids (wettable powders, dry flowables, liquid flowables and microencapulated) may be suitable for irrigation systems that have large application orifices and operate at higher pressures, but they may clog emitters in low-volume systems. Filtration may also limit the use of insoluble chemistries. If using cartridge filters and secondary filters, the solid particulates may collect on the mesh, which will reduce the applied rate of the chemical, reduce flow through the system, change the calibration, increase maintenance,and may also affect yield.
Before applying any chemistry, the system should be calibrated. Calibration identifies the amount of time it takes the chemistry to be delivered to the crop. To calibrate, some questions need to be answered. How large is each irrigated block (acres)? How much is required to be applied to the block (gallons, ounces)? These two questions refer to answering the amount and time to apply the product over a zone. In the following example, apply 5 pounds of nitrogen (N) per acre from liquid fertilizer 10-5-5. In 10-5-5, there is 10% N, 5% phosphate (P2O5; commonly referred to as P), and 5% potassium (K2O: commonly referred to as potash or K), at a specific gravity of ~10.0 pounds/gal. If the density is not given, weigh a known volume (mass/volume example: a gallon placed on a scale measuring pounds equals pounds/gallon). A simple way to identify how much nitrogen is in one gallon of 10-5-5 is as follows.
density x (10%N/100% = 0.10 N) = 10 pounds/gal x 0.10 N = 1 pounds N/gal
For two acres, 100 gallons of 10-5-5 will be applied during that irrigation set.
Acres in a block (2) x amount per acre to be applied (5 pounds N):
2A x (5 pounds N/A) x (1 gal/1 pounds N) = 10 gal of 10-5-5
Injection systems are manufactured with suggested settings. To ensure that the appropriate amount of chemical is being applied, a calibration should be conducted periodically. A basic method for calibration consists of using a vessel with volume graduations or using a known volume. Charge the irrigation system at standard operating pressures and allow it to irrigate for 30 mins. Place the suction line from the injector into your vessel filled with water. Measure the time it takes a known volume to draw into the system. Example, the system draws 16 oz of water in 2 min:
(16 oz/2 min) x (1 gal/128 oz) x (60 min/1 hr) = 3.75 gal/hr
With a known injection rate, the time to apply a chemical can be calculated:
10 gal x (hr/3.75 gal) = 2.66 hr or 2 hr 40 min
Applying pesticides is similar to applying soluble fertilizer. Fertilizer is mixed with water to form a solution that can be injected into the irrigation system. Pesticides that are labeled to be applied through an irrigation system will also be mixed with water. Mixing containers are available in many configurations, and it is important that the components are made of corrosion-resistant materials, are easy to maintain, and minimize exposure.
Irrigation and blueberry diseases
The timing, amount, and placement of irrigation can all have significant effects on the growth and spread of plant pathogens and the subsequent development of plant diseases in blueberry. Many fungal and oomycete pathogens capable of causing both foliar and root diseases of blueberry require water during the initial infection or spread from plant to plant. Furthermore, infection with some plant pathogens can exacerbate the effects of water stress on blueberry plants. All told, the proper irrigation of blueberry plants has the potential to profoundly impact the development of important blueberry pathogens, the severity of the diseases they cause, and the timing of symptom development.
Saturating the root area is well-known to promote infection by Phytophthora cinnamomi, the oomycete pathogen that causes Phytophthora root rot of blueberry. Abundant soil moisture directly promotes the production of reproductive structures known as sporangia, which release zoospores under moist conditions. Zoospores are motile “swimming” spores that can move through and be carried by soil or surface water until they reach root tissue and cause infection. Significant damage to the blueberry root system can result from P. cinnamomi infection, and damaged plants aresubsequently much more susceptible to drought stress. Due to the importance of water in this pathogen’s life cycle, avoiding oversaturation of irrigated soils and ensuring adequate drainage within field sites are key to avoiding root rot in blueberry plantings (Figure 12).
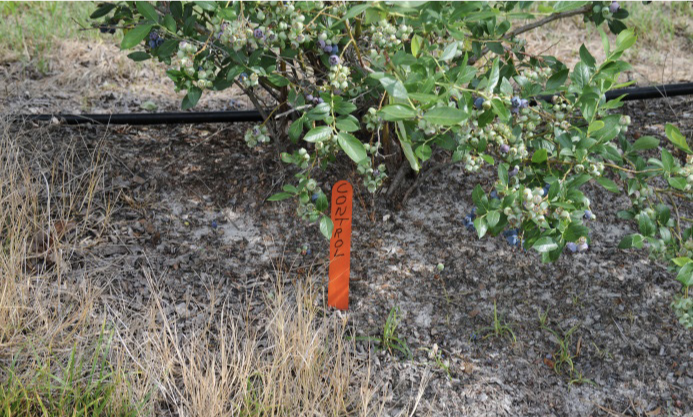
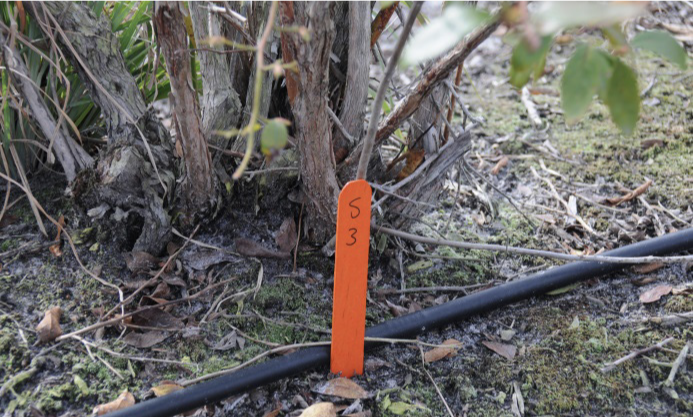
In addition, the life cycles of many fungal pathogens of blueberry capable of infecting blossoms, twigs, and leaves also rely on water for spread and infection. Septoria leaf spot and anthracnose ripe rot of blueberry both rely on splashing water (either from rain or from irrigation sources) to spread their spores within and between plants. Furthermore, free moisture on leaf surfaces also promotes fungal spore germination and infection. Accordingly, reducing irrigation volumes and optimizing delivery to reduce the presence of free moisture and promote rapid drying of the plant canopy can reduce the spread and severity of these pathogens in blueberry plantings.
Though not due to the direct effects of moisture (or lack thereof) on the pathogens themselves, plant drought stress can further impact the severity of some common blueberry diseases including bacterial leaf scorch and Botryosphaeriastem blight. Xylella fastidiosa, the cause of bacterial leaf scorch of blueberry, restricts plant water and nutrient flow by colonizing the xylem vessels of blueberry host plants. As a result, bacterial leaf scorch symptoms may appear more pronounced on infected plants experiencing drought stress. Increased bacterial leaf scorch severity may be more readily evident on southern highbush cultivars, such as ‘Star’, which seem prone to drought-stress symptoms. Likewise, though infection of blueberry plants with Botryosphaeria may occur at any time, the onset of Botryosphaeria stem blight symptoms often corresponds with periods of drought or heat stress.
Suggestions on irrigation
Throughout the growing season, crop demand for water varies. Factors such as plant age, time of the year, heat, humidity, amount of light, soil type, and rainfall can affect transpiration rates of water moving out of the soil, through the plant, and into the atmosphere. Evapotranspiration (ET) is a term that describes water loss as evaporation and transpiration. Water evaporation is the transition from liquid to gas and is lost directly to the atmosphere from soil and plant surfaces. Transpiration is the movement of water through a plant and its release as a gas. Irrigation by ET is based on water loss from the leaf surface and the soil surface. For ET-based irrigation, it is assumed that the soil is well-irrigated and water loss from the soil has minimal to no impact on the water/plant relationship. To identify the rate of water usage, reference evapotranspiration (ETo) is used as a factor that represents water movement through a green crop that is completely shading the ground and of uniform height in well-irrigated ground. This is either associated to grass or alfalfa depending on the crop to be irrigated, for example, turf or blueberries. From field observations, crop coefficients (Kc) are developed for specific crops that include growth stages and seasonal variations in Kc. The calculation of crop evapotranspiration (ETc) is ETo x Kc. As a basis for irrigation, ET has been used for irrigation as web-based apps and modeling programs, where weather data, Kc, and plant age are inputs that inform the user when and how much water to apply. This is determined with one critical known value, a well-irrigated soil profile.
Soil moisture monitoring
Using ET to irrigate is effective, but keeping optimum levels of moisture in sandy soils may be a challenge, which necessitates soil moisture monitoring. Soils that are sandy have high infiltration rates and moisture depletes under peak water usage when windy and during drought. Soil moisture monitoring is another effective method to irrigate. Using this method, soil moisture content is related to plant status. Irrigation scheduling is directly related to soil moisture.
The clod method is commonly used to identify soil moisture without any instruments. Dig into the soil (for blueberry, 6 to 8 inches is sufficient) and extract a fistful of soil, squeeze it in your palm, and observe whether water oozes between your fingers. Then open your hand and look at the clod. If water oozes freely with minimal pressure, then the soil is at 75% to 100% moisture, and there’s no need to irrigate. If water oozes with great pressure and forms a clod that sticks together, the soil moisture is 50% to 75%, and it’s time to irrigate. If no water oozes and forms a poor clod, then the soil is below the wilting point and irrigation is past due. This method is effective but can be easily misinterpreted, which may cause losses in production.
Three types of instrumental soil moisture monitoring devices are commonly used: tensiometers, electrical resistance blocks, and capacitance or dielectric soil moisture sensors. Tensiometers do not require an electrical source toread soil moisture. These devices measure soil water tension and consist of a plastic pipe with a ceramic cup inserted into the soil and a vacuum gauge attached at the other end to read soil tension (Figure 13). For blueberry, the depth of the soil moisture probe should be 6 to 8 inches. Observations conducted at the UGA Blueberry Farm in Alapaha, Georgia, showed that soil moisture monitoring at 4 to 8 inches was sensitive to plant water uptake, irrigation, and rain events. At 12 inches, soil moisture readings showed little response over the trials.
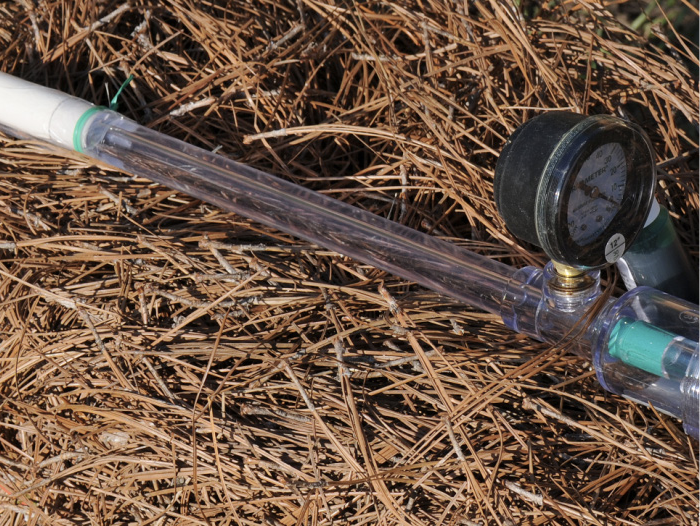
Tensiometers must be preconditioned before being deployed. The ceramic cup is porous and should be soaked in water overnight. More specifically, the device should be filled with a water and dye solution and the ceramic cup should be immersed in water with a vacuum drawn. This should be repeated numerous times until there are no more visible bubbles in the probe. The plastic pipe can be purchased in varying lengths and is close to 13/16 of an inch in diameter. A soil probe or a 3⁄4-inch metal pipe can be used to bore a hole into the soil. The bore should be marked at 6 to 8 inches so that the probe can be placed into the soil at the proper depth. A slurry of water and native soil should be made in a bucket, and a bit of slurry should be poured into the hole. A vacuum pump should then be used to draw air from the device, and it will take 24 hours for the device to equilibrate. Note that in sandy and rocky soils, a good seal between the soil and the ceramic cup may not be possible. Without good soil-to-ceramic contact, soil moisture cannot be determined. Although tensiometers do not need electricity to power the system, they can be fitted to automate an irrigation system.
Electrical resistance blocks or gypsum blocks are inexpensive soil moisture monitoring devices. The probes can be placed into the soil for long periods of time with minimal wear; however, wires are vulnerable to wildlife damage and should be placed in protective enclosures from below the soil line to the meter. The probes measure resistance and need an electrical source, which is provided by a battery in the meter or from a base station as pictured in Figure 14. These probes do not measure the amount of moisture but are a reliable indicator of water availability to the plant. As reviewed previously, soil type is very important, and sandy soils should be irrigated when soil moisture content is 10 to 20 centibars or kilopascals. Converting centibars to percent available water in the soil is dependent on soil type (Figure 15). For example, when the probe reads 20 centibars in loamy sands, there is 62% available water in the soil. This is at a level where water stress will become apparent in blueberry if not corrected.
Blueberries are grown in acidic soil (4.0 to 5.2 pH), and under these conditions gypsum blocks can dissolve over time. This is a slow process that may take two to three years, however, adding sulfuric acid to the irrigation water may shorten the life of the probe faster than if irrigated with nonacidified water.
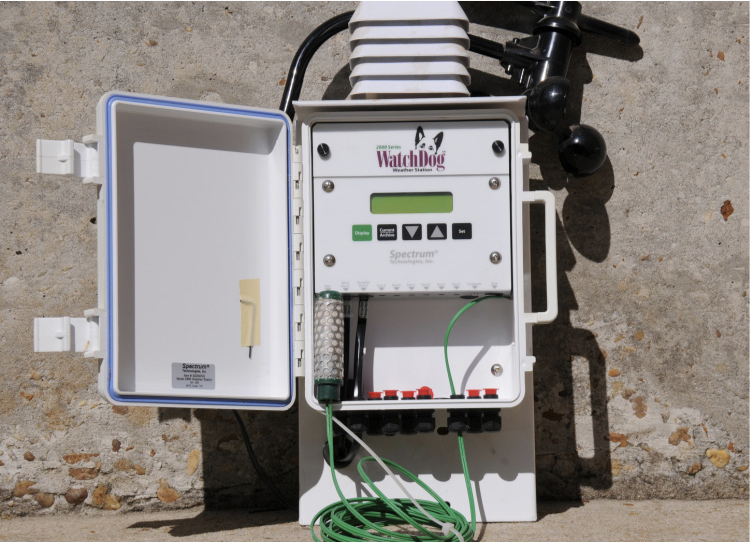
There are many field configurations to follow for soil moisture monitoring with electrical resistance blocks. If your irrigation zones have different soil types, place probes in soil that represents the majority of the irrigation zone. Place the probe within the wetting area of the irrigation system, within the root zone, and at a depth where the majority of roots populate. For blueberries, the probe should be located 6 to 8 inches in depth, within 12 inches from the crown of the plant, and no more than 2 inches from an emitter of a dripline. Installing probes requires a similar process to tensiometers: soak the probes in water overnight and test prior to installation. In the blueberry bed, drill a hole into the soil at the appropriate diameter and depth. Make a slurry of waterand soil in a bucket, place a small amount of the slurry into the hole, and place the probe into the hole. To accomplish this, use a piece of pipe with an outside diameter that is less than the diameter of the probe, run the wire through the pipe, and gently push the probe into the hole. Use the slurry to fill the hole, and soak the hole with water. Animals will nibble the wires, so to protect them, either bury or run the wires through conduit, like PVC pipe. If the soil is to be monitored with a portable meter, coil the wire into the pipe and cap it. If running to a weather station or automated irrigation station, protect the wire all the way to the station.
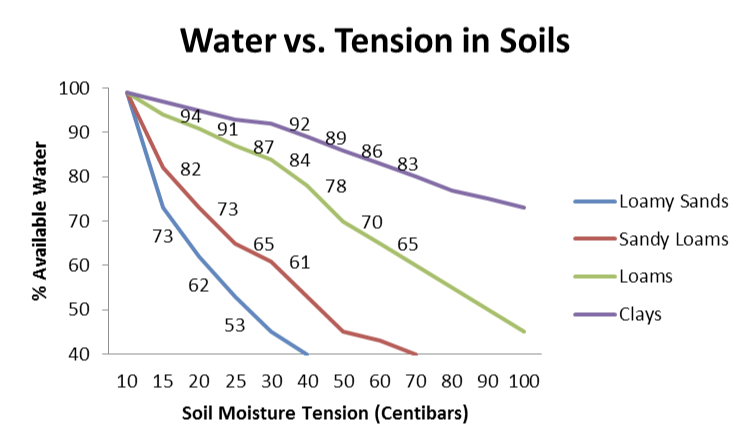
Once the probes are deployed, take initial readings. If the soil is sandy and the probes read percent, irrigate at 90% to 80% soil water content or at 10 to 20 centibars. After irrigating, check the probes—they should read >90% or <10 centibars. If they are not responding, there could be poor soil probe contact, the probe could be set too far away from an emitter, or it could have been damaged during installation. If they’re working correctly, take readings prior to the next irrigation. If the readings show a water deficit, then the placement is appropriate. If the readings have only slightly changed, there may be no need to irrigate or the probes may be buried too deep. To identify the soil status, dig into the soil away from the probe but under the drip line to a 6-8 inch depth, then squeeze a handful of soil to determine whether there is sufficient moisture (see clod method above for levels).
Dielectric soil moisture sensors measure soilmoisture by measuring capacitance or the velocity of an electromagnetic wave or pulse as it passes through the soil (Figure 16). Dielectric soil sensors are used in fine to coarse soils; however, the sensors need calibration. Generally, the manufacturer will provide a universal calibration but in some instances a site-specific calibration needs to be performed. If the sensor reading is above 50% after irrigating or when the soil is at field capacity, this is an indication the sensor should be calibrated. Additionally, the field capacity volumetric moisture content should be compared with sensor values. If the readings are significantly different, then a site calibration should be performed. To perform a site- specific calibration, a calibration curve needs to be executed. This curve is developed by collecting native soil at the planting, drying the soil, adding known volumes of water to the soil, and measuring the soil moisture with the probes.
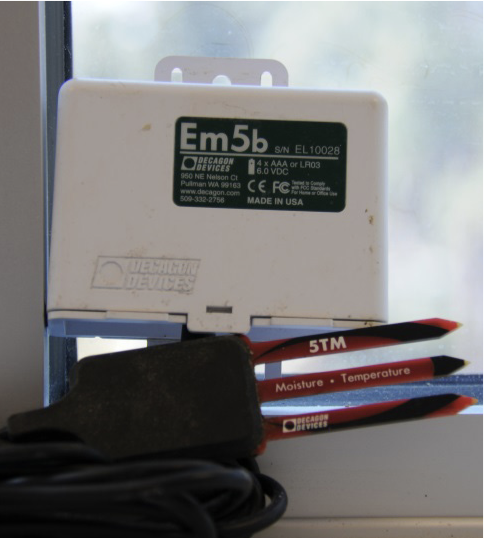
Dielectric soil moisture sensors usually have two to three forks that are placed into the soil vertically. Dig a hole into the soil with an auger, retain the soil, and set some aside to make a soil/water slurry. The size of the hole should accommodate the probe and your hand. At the appropriate depth, 6-8 inches for blueberry, the forks are inserted into the soil (vertically, top-to-bottom probe alignment). Air gaps between the soil and the sensor will give erroneous readings, so pay careful attention to sensor placement to ensure accuracy. Backfill the hole with the extracted soil, and if the sensor seems loose or there is poor soil-to-probe contact, make a pocket exposing the probe base and fill the pocket with the soil slurry. Finish filling the hole, then place the wire beneath the soil surface, protecting the wire from the soil to the base station or data logger. Once the system is in place, soak the area where the probe is positioned. Soil moisture readings are ready to measure.
Dielectric soil moisture monitors are not as sturdy as tensiometers and gypsum blocks, so handle them with care. They should not be forced into the soil or yanked out of the soil by their wire. Probes may not work well in saline soils and finely textured soils (clays). Read the instructions thoroughly before purchasing and know the soil type into which the probe will be deployed. This can prevent mistakes that may lead to misinterpreting water demand.
References:
Baum M. C., Dukes, M. D., & Haman, D. Z. (2015). Selection and Use of Water Meters for Irrigation Water Measurement. University of Florida Extension, EDIS, ABE18 p 1-6.
Brannen, P. M., Chang, C-J., Boland, R. T., Horton, D. L., & Krewer, G. W. (2016). Bacterial Leaf Scorch of Blueberry. University of Georgia Extension Circular 922. 1-6.
Harrison, K. (2012). Drip Irrigation for Pecans. University of Georgia Extension Bulletin 936. 1-12.
Harrison, K. (2012). Chemigation in Georgia. University of Georgia Extension Bulletin 1298. 1-8.
Harrison, K., Porter, W. M., & Perry, C. (2015). Factors to Consider in Selecting a Farm Irrigation System. University of Georgia Extension Bulletin 882. 1-12.
Martin, D. L., Dorn, T. W., Melvin, S. R., Corr, A. J., & Kranz, W. L. (2011). Evaluating Energy Use for Pumping Irrigation Water. Proceedings of the 23rd Annual Central Plains Irrigation Conference, Burlington, CO. Feb. 22-23. 104-116.
Pennisi, B., & Kessler, R. (2012). Fertilizing Injectors. University of Georgia Extension Bulletin 1237. 1-16.
Prestwich, C., Walker, J., Robinson, P., Busch, J., & Sampson, R., eds. (2016). National Engineering Handbook: Chapter 11 Sprinkler Irrigation. USDA NRCS, 210-VI-NEH, Amendment 80, Aug. 1. 1-200.
Polashock, J. J., Caruso, F. L., Averill, A. L., & Schilder, A. C., eds. (2017). Compendium of Blueberry, Cranberry, and Lingonberry Diseases and Pests, 2nd Edition. The American Phytopathological Society. St. Paul, MN, USA.
Rogers, D. H., Lamm, F. R., Alam, M., Trooien, T. P., Clark, G. A., Barnes, P. L., & Mankin, K. (1997). Efficiencies and Water Losses of Irrigation Systems. Kansas State Cooperative Extension Service, MF-2243.
Schwankl, L. J. (2018). Irrigation Scheduling. University of California, UC Drought Management. Accessed Mar. 3, 2018. Retrieved from http://ucmanagedrought.ucdavis.edu/Agriculture/Irrigation_Scheduling/
Sumner, P. E., & Bader, M. J. (2012). Pesticide Storage and Mixing Facilities. University of Georgia Extension Bulletin 1095. 1-16.
Status and Revision History
Published on Oct 22, 2018